
[ad_1]
Generation of self-renewing neural crest-derived progenitor cells expressing cartilaginous progenitor and stem cell markers from hPSCs
Human pluripotent stem cells (hPSCs) were cultured on Matrigel-coated surfaces in an E8 medium. Neural crest (NC) was induced by switching from hPSC growth medium to NC induction medium consisting of DMEM/F12, N2, B27, 20 ng/ml BMP4 and 2 μM the TGF-β receptor inhibitor SB431542 for 7 days. Previously, we successfully self-renewed primitive neural stem cells derived from hESCs by using a medium containing the glycogen synthase kinase-3 (GSK-3) inhibitor CHIR99021, SB431542, and human leukaemia inhibitory factor22. In attempting to induce the self-renewal of this BMP4- and SB431542-induced NC population, we fortuitously defined a culture medium formulation that could stably maintain the self-renewal of this NC population. The medium consisted of DMEM/F12 supplemented with N2, B27, 1 μM CHIR99021, 2 μM SB431542, 0.1 μM smoothened agonist (SAG), 20 ng/ml epidermal growth factor (EGF), 20 ng/ml fibroblast growth factor 2 (FGF2), and 1 μM BMP receptor inhibitor (DMH1), and is hereafter referred to as CSSEDF (Fig. 1a). RNA-seq was performed for five hPSC cell lines, including H1, Hues9, H9, Hues7 and ihPSC (ATCC-DYR0100), on Days 3, 7, 16 and 30 after induction. Principal component analysis (PCA) suggested that the induction protocol was highly reproducible across different hPSC lines (Fig. 1b). Immunostaining showed that NC markers, such as AP2, DLX2, and ETS123, were uniformly expressed in hPSCs treated with BMP4 and SB431542 for 7 days (Supplementary Fig. 1a), suggesting the induction of NC cells. RNA-seq analysis at different induction points showed that markers representing neural ectoderm and NC were upregulated sequentially during the induction process (Fig. 1c, d)24. Real-time PCR analysis revealed a rapid loss of OCT4, NANOG, and SOX2 expression after treatment with BMP4 and SB431542 for 7 days (Supplementary Fig. 1b). However, the expression of neural ectodermal and neural plate border markers, SIX1, TUBB3 and ZIC125,26,27, was upregulated after induction. In addition, the expression of the mesodermal markers KDR, TBX6, and PAX128,29,30 and the endodermal markers GATA4, GATA6, and SOX731,32 were not significantly induced after treatment with CSSEDF medium (Supplementary Fig. 1b). All these data suggest the successful generation of NC populations from hPSCs after 7 days of treatment with BMP4 and SB431542.
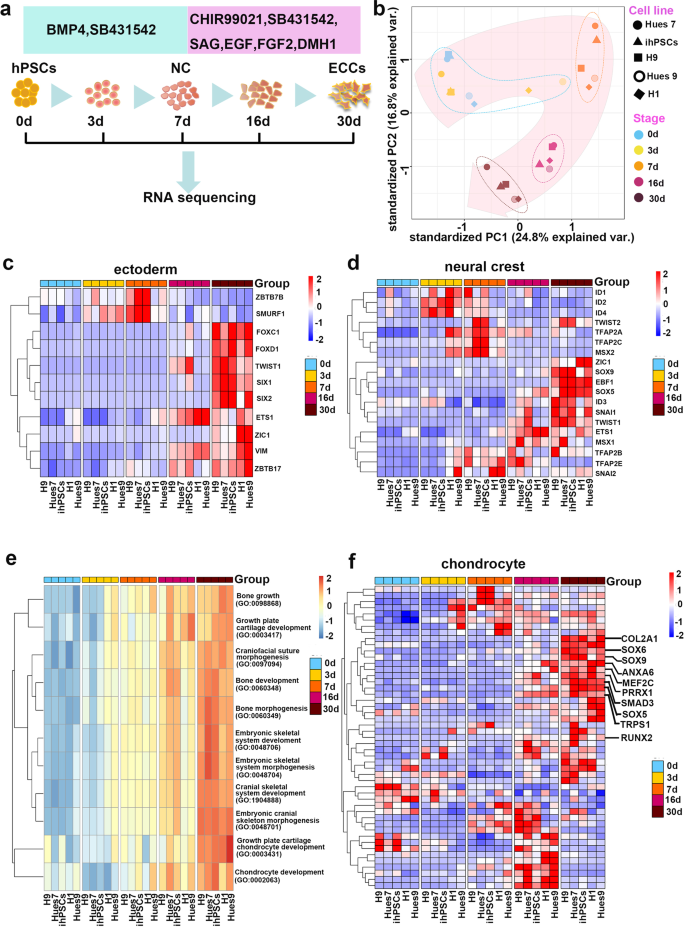
a Schematic representation of the NC-derived progenitor cell induction process. b PCA indicates that five unique hPSC (H1, Hues9, H9, Hues7 and ihPSC) lines followed similar differentiation trajectories. c Genes encoding ectoderm markers were upregulated during induction. d Genes encoding NC markers were upregulated during induction. e GO analysis highlighted bone and cartilage development, especially cranial skeleton morphogenesis and cranial skeletal system development, in NC-derived progenitor cells. f Genes encoding chondrogenesis markers were upregulated during induction.
After treatment with CSSEDF medium, the cell morphology gradually changed to mesenchymal monolayers (Supplementary Fig. 1c, d). To determine the nature of CSSEDF-expanded cells, gene ontology (GO) enrichment analysis of the genes using the R package GAGE was performed. Significantly upregulated GO terms in biological processes highlighted bone and cartilage development, especially cranial skeleton morphogenesis and cranial skeletal system development (Fig. 1e). We next compared the TCGA transcriptome data between cells at different induction stages to determine the differentially expressed genes. The top 10 upregulated and downregulated genes are listed in Supplementary Fig. 2a. Consistently, gene set enrichment analysis (GSEA) showed a significant upregulation of the cranial skeleton and cartilage gene set during induction (Supplementary Fig. 2b). Chondrogenic markers such as SOX5, SOX6, SOX9, RUNX2, COL2A1, ANXA6, MEF2C, PRRX1, SMAD3, and TRPS1 were upregulated after induction for 30 days (Fig. 1f)14,33,34,35,36,37. PCR analysis revealed that the expression of the chondrogenic genes SOX9, ACAN, RUNX2, SP1, and OCN was dramatically upregulated during the induction process (Supplementary Fig. 2c). Taken together, these data suggest that our dual-phase induction may produce an ectodermal chondrogenic lineage.
CSSEDF can sustain the long-term self-renewal of these cells on Matrigel-coated surfaces. CSSEDF-expanded cells were routinely passaged at 1:10 and cultured for >30 passages without obvious loss of proliferative capacity. Single cells at passage 5 and passage 16 were clonogenic on Matrigel in the presence of CSSEDF (Fig. 2a and Supplementary Fig. 3a). Immunostaining showed that neural ectodermal markers, including SIX1, NESTIN and ETS126,38, were positive in the CSSEDF-expanded cells (passage 5; Fig. 2b–d), which is consistent with their NC origin. Immunostaining revealed that both early-passage (passage 5) and late-passage (passage 18) CSSEDF-expanded cells stably expressed genes identified as cartilaginous progenitor/stem cell (CPC/CSC) markers, including SOX9, RUNX2, SOX5, TWIST1, and CD29 (Fig. 2e–i and Supplementary Fig. 3b-f)39. SOX9 is a universally accepted marker of chondrogenesis. However, SOX9 does not work alone, and its function depends on the coexpression of SOX5/640. RUNX2 is a master regulator of chondrocyte specification and differentiation39. TWIST1 can inhibit the differentiation of CPCs/CSCs into downstream cell types and maintain the characteristics of CPCs/CSCs41. CD29 is reported to be most highly expressed in CPCs and plays an important role in the chondrogenic differentiation and cartilage tissue formation of CPCs42. These results suggest that CSSEDF-expanded cells share CPC/CSC markers. Notably, immunostaining confirmed the expression of genes recently identified as neural crest-derived TMJ condylar cartilage markers43, such as FOXC1, FOXC2 and MSX1, in CSSEDF-expanded cells (Fig. 2j–l). The stable phenotype of CSSEDF-expanded cells after extensive passages was further confirmed by flow cytometry. Both early-passage (passage 9) and late-passage (passage 19) CSSEDF-expanded cells exhibited nearly identical expression patterns for a set of chondrocyte-specific markers, such as SOX5 (98.8% and 95.9% positive), SOX9 (97.0% and 97.6% positive), TWIST1 (94.4% and 93.4% positive), and CD29 (98.6% and 98.4% positive) and the cell proliferation marker Ki-67 (83.3% and 84.2% positive; Fig. 2m). PCR further demonstrated that early-passage (passage 8) and late-passage (passages 19 and 25) CSSEDF-expanded cells stably expressed genes identified as chondrogenic markers, including SOX9, COL1A1, SOX5, COL2A1, OCN and RUNX2 (Fig. 2n).
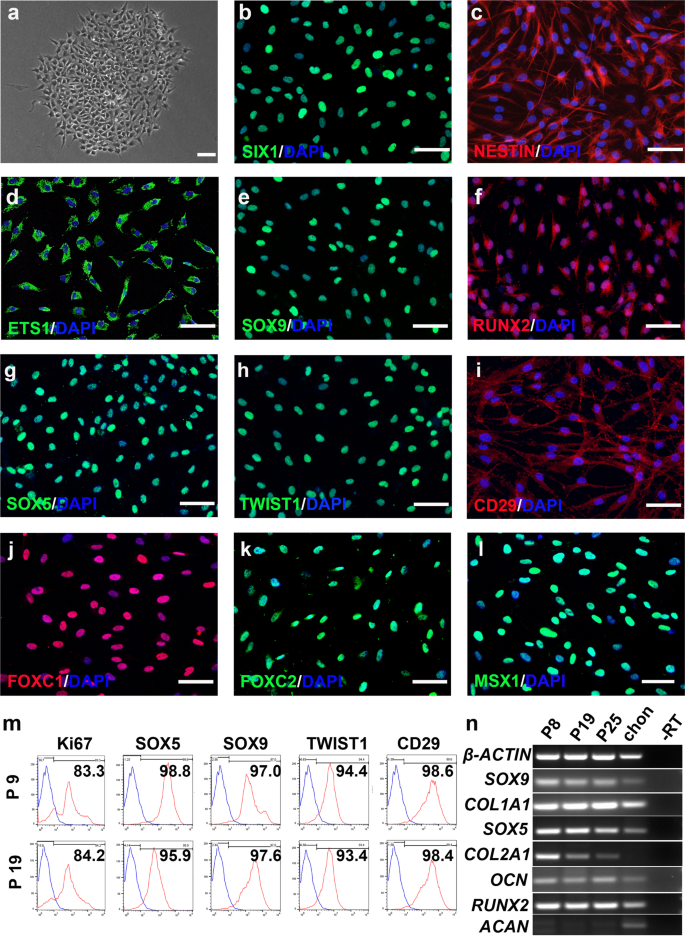
a A single CSSEDF-expanded cell (passage 5) colony on a Matrigel-coated surface. Scale bars, 50 µm. b–d Immunocytochemistry showing that CSSEDF-expanded cells expressed genes identified as neural ectodermal markers, including SIX1, NESTIN and ETS1. Scale bars, 50 µm. e–i Immunocytochemistry showing that CSSEDF-expanded cells (passage 5) expressed genes identified as CPC/CSC markers, including SOX9, RUNX2, SOX5, TWIST1, and CD29. Scale bars, 50 µm. j–l Immunocytochemistry showing that CSSEDF-expanded cells (passage 5) express genes recently identified as TMJ condylar cartilage markers, including FOXC1, FOXC2 and MSX1. Scale bars, 50 µm. m Flow cytometry analysis showing that CSSEDF-expanded cells stably express cell proliferation and CPC/CSC markers after long-term in vitro expansion. n Gene expression of chondrocytic lineage markers by CSSEDF-expanded cells at different passages and patient TMJ condylar cartilage were analysed by PCR. CPCs/CSCs: cartilaginous progenitor cells/cartilaginous stem cells.
CSSEDF-expanded cells have a propensity to differentiate into chondrocytes
Next, we evaluated the spontaneous differentiation of CSSEDF-expanded cells in N2B27 basal medium free of CSSEDF self-renewal supplements in the absence of any inducing agents. CSSEDF-expanded cells were evaluated in monolayer, collagen sponge and suspension culture conditions. Under monolayer conditions, the cells stopped proliferating. Scattered white flakes were visible in the Petri dish. Haematoxylin and eosin (HE) staining of these flakes revealed that cartilage lacuna was formed and that the ECM was rich after 8 weeks in monolayer culture (Supplementary Fig. 4). We further evaluated the expression of osteogenic differentiation markers ALPL, SPP1, and SP7, and adipogenic differentiation markers FABP4, CEBPA and APN in CSSEDF-expanded cells on Day 0 and Week 8 after spontaneous differentiation under monolayer condition. RT-PCR analysis showed that the expression of osteogenic and adipogenic differentiation markers was virtually undetectable (Supplementary Fig. 5). These data confirmed that CSSEDF-expanded cells were specifically chondrogenic, and these cells are hereafter referred to as ectodermal chondrogenic cells (ECCs).
ECCs were seeded onto a collagen sponge and cultured with N2B27 medium. After 4-week culture, the cells in the sponge were largely immature chondrocytes (Supplementary Fig. 6). At 8 weeks, the collagen sponge developed a smooth and glistening appearance (Fig. 3a). Panoramic scanning of HE staining showed scattered cartilage masses in the collagen scaffold. Chondrocytes were large and buried in the cartilage lacuna with rich ECM (Fig. 3b) and strong positive toluidine blue, alcian blue and safranin O staining, indicative of proteoglycan-rich cartilage-like ECM (Fig. 3c–e). The cartilage masses expressed collagen II, collagen X, aggrecan and RUNX2, as expected (Fig. 3f-i). Moreover, collagen I was also detected, consistent with its known expression in mandibular condylar chondrocytes (Fig. 3j). Interestingly, lubricin, a heavily O-glycosylated protein44 that is essential for boundary lubrication of articular cartilage, was also detected (Fig. 3k). ECCs were also cultured in suspension using ultralow attachment plates. The cells formed spheres in CSSEDF medium after 5 days. The medium was then changed to N2B27, and the growth of spheres slowed significantly, with spheres adopting a glistening appearance (Supplementary Fig. 7a). Panoramic scanning showed homogenous chondrocytes with rich ECM in the sphere, but the cells and cartilage lacuna were smaller than the chondrocytes differentiated in the collagen sponge (Supplementary Fig. 7b). Sphere tissues showed strong toluidine blue and alcian blue staining, while safranin O staining was weak. The sphere tissue also demonstrated strong expression of collagen II, collagen X and collagen I (Supplementary Fig. 7c–h).
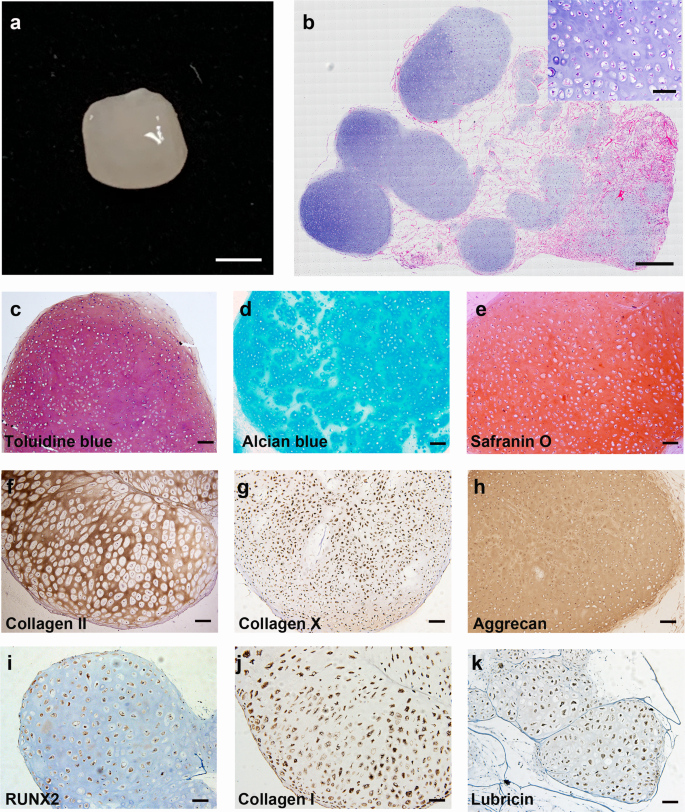
a Gross appearance of ECCs seeded in a collagen sponge for 8 weeks. Scale bars, 1 mm. b Panoramic scanning showing the formation of cartilage masses in the collagen scaffold (inset: HE staining enlargement). Scale bars, 200 µm. (Inset) Scale bars, 50 µm. c–e The chondrocytes were positive for toluidine blue, alcian blue and safranin O staining. Scale bars, 100 µm. f–k Immunohistochemical staining showing that the chondrocytes express common chondrocytic markers, including collagen II, collagen X, aggrecan, RUNX2, collagen I, and the articular cartilage marker lubricin. Scale bars, 100 µm.
To further characterize the differentiation potential of hPSC-derived ECCs, a collagen sponge seeded with ECCs and cultured for 4 weeks was transplanted subcutaneously into the dorsum of NOD-SCID mice. Histological analysis confirmed no teratoma formation in the transplanted sponge at 4 weeks (Fig. 4a). Strong positive safranin O, alcian blue and toluidine blue staining in the sponge indicated proteoglycan-rich cartilage-like ECM (Fig. 4b–d). The generated cartilage tissue expressed collagen II, collagen X, aggrecan and RUNX2 (Fig. 4e–h). Collagen I and lubricin were also detected in the grafts, which was similar to the staining pattern found in the tissue in vitro (Fig. 4i, j). Together, these results demonstrate that ECCs can spontaneously differentiate into chondrocytes both in vitro and in vivo after the withdrawal of self-renewal factors.
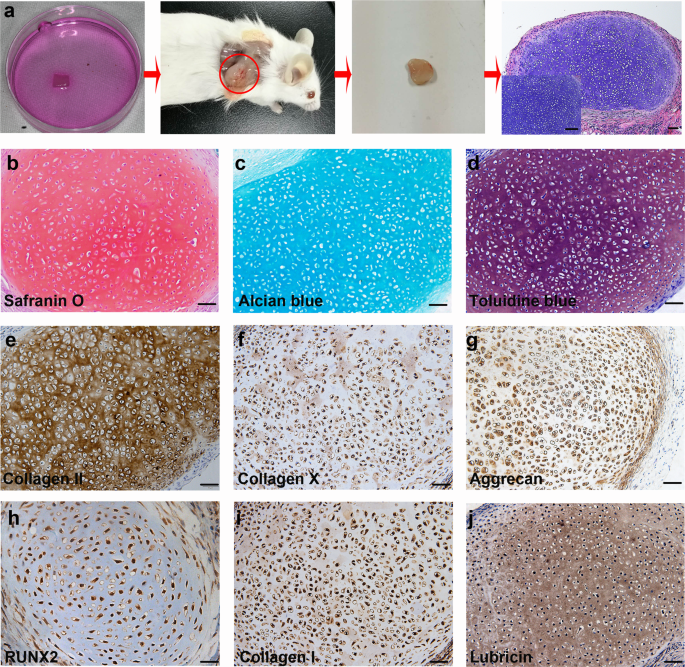
a Schematic representation showing ECCs seeded in a collagen sponge in vitro for 4 weeks and surgically transplanted subcutaneously into the dorsum of NOD-SCID mice. The implants were harvested after 4 weeks and histologically confirmed to be cartilage. (Inset) Enlargement of HE staining. Scale bars, 200 µm. (Inset) Scale bars, 50 µm. b–d Harvested cartilage was positive for safranin O, alcian blue and toluidine blue staining. Scale bars, 100 µm. e–j Immunohistochemical staining showing that differentiated chondrocytes express common chondrocytic markers, including collagen II, collagen X, aggrecan, RUNX2, collagen I, and the articular cartilage marker lubricin. Scale bars, 100 µm.
ECCs have similar single-cell transcriptome profiles to TMJ condylar chondrocytes
The neural crest contributes to early craniofacial development by generating mesenchymal tissues, including chondrocytes. As noted, ECCs expressed the genes recently identified as neural crest-derived TMJ condylar cartilage markers, such as FOXC1, FOXC2 and MSX1. Next, single-cell transcriptome profiles of TMJ condylar chondrocytes (TMJ-CC) and ECCs were acquired. Identification of subpopulations showed that TMJ-CC were comprised of four clusters of chondrocytes (chondrocyte 1, n = 7688, 62.1%; chondrocyte 2, n = 2205, 17.8%; chondrocyte 3, n = 2074, 16.7%; and chondrocyte 4, n = 304, 2.4%) and a small cluster of monocytes (n = 115, 0.9% of the total population; Fig. 5a). Both TMJ-CC and ECCs were enriched in the mandibular condyle and NC markers, such as COL1A1, BARX1, FOXC1, MSX1, SIX1, and TWIST1 (Fig. 5a, b), indicating that TMJ-CC and ECCs exhibited similar developmental origins.
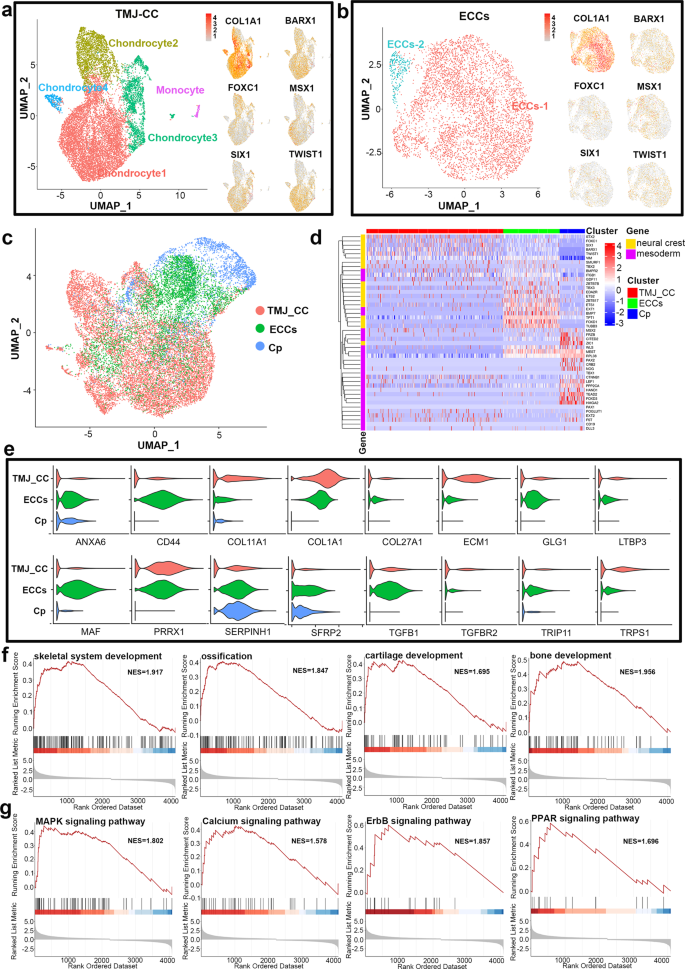
a and b Both TMJ-CC and ECCs were enriched in mandibular condyle and neural crest markers, such as COL1A1, BARX1, FOXC1, MSX1, SIX1, and TWIST1. c UMAP confirmed that ECCs are closer to TMJ-CC than Cp from hiPSCs via the paraxial mesoderm. d Both the TMJ-CC and ECCs exhibited high expression of neural crest markers, while genes involved in mesoderm were clustered in the Cp. e Violin plots showing that TMJ-CC and ECCs presented nearly the same chondrogenic markers. f GSEA suggesting that ECCs had different gene expression from Cp in skeletal system development, ossification, cartilage development, and bone development. g MAPK, calcium, ErbB, and PPAR signalling pathway-associated genes were different between ECCs and Cp.
Next, we compared ECCs, TMJ-CC and chondroprogenitor (Cp, a lineage from hiPSCs via the paraxial mesoderm)45 based on single-cell data. Single-cell RNA-seq data were subjected to unsupervised clustering and visualized using uniform manifold approximation and projection dimension reduction analysis (UMAP). We observed that ECCs are closer to TMJ-CC than Cp (Fig. 5c). Notably, both the TMJ-CC and ECCs exhibited high expression of NC markers, while genes involved in mesoderm were clustered in the Cp (Fig. 5d), which was in accordance with their trajectories. Compared with Cp, violin plots showed that TMJ-CC and ECCs presented nearly the same chondrogenic markers (Fig. 5e). Although they were both chondroprogenitors, GSEA suggested that ECCs had different gene expression from Cp on skeletal system development, ossification, cartilage development, and bone development (Fig. 5f). We also observed the distribution and enrichment score of MAPK, calcium, ErbB, and PPAR signalling pathway-associated genes between the ECCs and Cp (Fig. 5g). The top 10 significantly differentially expressed (SDE) genes of TMJ-CC, ECCs and Cp were further represented in the heatmap shown in Supplementary Fig. 8a. GO analysis of these dynamically expressed genes indicated that cranial suture morphogenesis, craniofacial suture morphogenesis, and cartilage development involved in endochondral bone morphogenesis were enriched in both ECCs and TMJ-CC (Supplementary Fig. 8b). Kyoto Encyclopaedia of Genes and Genomes (KEGG) analyses of these dynamically expressed genes indicated that neurotrophin, TGF-beta, calcium, insulin, and JAK-STAT signalling pathways were remarkably enriched in major clusters of TMJ-CC and ECCs (Supplementary Fig. 8c).
To further evaluate the profile of ECCs, we compared TMJ-CC with the cells differentiated for 28 days from ECCs and Cp by single-cell transcriptome analysis. Interestingly, a cluster of cells in ECC-28d overlapped with TMJ-CC, while only a few cells in Cp-28d were close to TMJ-CC (Supplementary Fig. 9a). Further clustering analyses yielded 7 major clusters of cell types (Supplementary Fig. 9b). Clusters 1–4 were chondrocytes, and Clusters 5, 6 corresponded to progenitor cells. Cluster 7 was monocytes from TMJ-CC. Both ECC-28d and TMJ-CC expressed fibrocartilaginous markers (e.g., COL3A1 and COL1A2), cranial chondrocyte markers (e.g., GNAS, BARX1, MGP)46,47,48, and craniofacial markers (e.g., DCN, ITGB1)49,50. Cp-28d mainly expressed hypertrophic chondrocyte markers in the growth plate (e.g., CYR61, CXCR4, CTGF)51,52, primary chondrocyte markers (e.g., GPX1)53 and mesoderm markers (e.g., MESDC2)54 (Supplementary Fig. 9c).
hPSC-derived ECCs can be used to repair cartilage defects
Cartilage defects are common disorders that mainly affect articular cartilage. hPSC-derived ECCs provide an opportunity to repair defective TMJ condylar cartilage derived from the CNC. However, it is difficult to establish a TMJ cartilage repair model in rats. Thus, we used a rat knee cartilage defect model to test the ability of hPSC-derived ECCs to repair articular cartilage. A full-thickness rat cartilage defect was created in each knee of rats. A collagen sponge with or without ECCs was implanted into the right or left knee defect, respectively (Fig. 6a). In the ECC group, the regenerated tissue fully filled the defect, smoothly integrated with the host cartilage, and presented a white glistening appearance. Green fluorescence was observed in the regenerated tissue, and the fluorescence exactly matched the repaired tissue under the fluorescence stereoscope (Fig. 6b). In the control group, damaged cartilage exhibited scarring with apparent poor regeneration. No green fluorescence was detected in the harvested tissue (Fig. 6c). Immunofluorescent staining showed that green fluorescent protein (GFP) expression was positive in the transplanted site 4 weeks later (Fig. 6d). Accordingly, no GFP was detected in the control group (Fig. 6e).
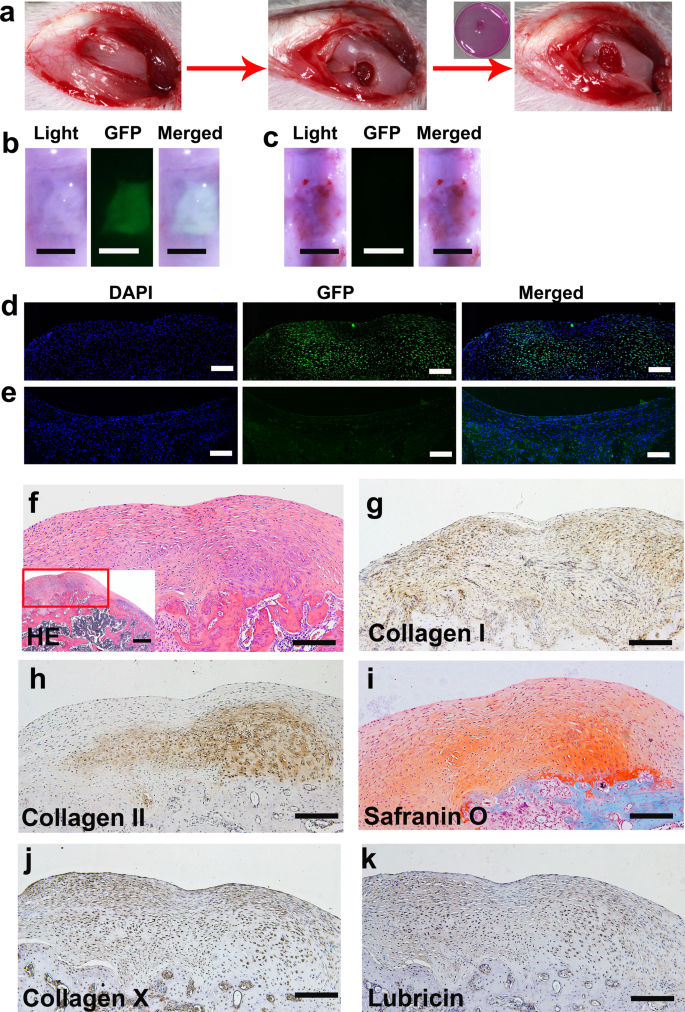
a Schematic representation showing that a full-thickness rat cartilage defect was created. ECCs were seeded in collagen sponges, cultured in vitro for 4 weeks, and then surgically transplanted into cartilage defects. b, d Knees treated with GFP-expressing ECCs loaded on a collagen sponge were harvested 4 weeks after transplantation. GFP expression was detected in the ECC group. b Scale bar, 1 mm. d Scale bar, 50 μm. c, e No GFP expression was observed in the control group transplanted with only the collagen sponge. c Scale bar, 1 mm. e Scale bar, 50 μm. f HE staining showing that the repaired tissue was cartilage-like. (Inset) HE staining at low magnification. Scale bar, 100 μm. (Inset) Scale bar, 200 μm. g Immunohistochemical staining revealing that collagen I was present throughout the cartilaginous cell layers. h Immunohistochemical staining showing that collagen II was predominant in the deep layer. i Safranin O is more significantly positive than the deeper layer. j, k Immunohistochemical staining showing that collagen X and lubricin are expressed in the regenerated cartilage tissue. g–k Scale bar, 100 μm.
Mature TMJ condylar cartilage consists of the following four zones (Supplementary Fig. 10a)16, which are different from knee cartilage (Supplementary Fig. 10b): (1) articular surface of fibrous tissue expressing collagen I, (2) proliferative cells in the prechondroblastic zone expressing collagen I, (3) a chondroblastic zone expressing collagen II, proteoglycans aggrecan, decorin, chondroitin sulfate PG, and keratin sulfate PG, and (4) a hypertrophic zone adjacent to bone expressing collagen X. On the other hand, knee cartilage, as typical hyaline cartilage, demonstrates homogenous ECM, which is dominated by collagen II. Interestingly, in the ECC group, the regenerated tissue became part of a cartilage-like TMJ condyle with an obvious layered structure (Fig. 6f). The ECCs formed cartilage, as evidenced by collagen I expression throughout the cartilaginous cell layers, whereas collagen II and safranin-O staining for chondroitin sulfate PG were observed predominantly in the deep layer. Collagen X was detected both in the super and deep layers. Lubricin was positive in the regenerated cartilage tissue (Fig. 6g–k). In the control group, the scar tissue was thinner and consisted of fibrocytes with vascular invasion. Safranin-O staining and collagen I and collagen II immunofluorescence costaining showed the absence of chondrocyte ECM (Supplementary Fig. 11).
The above data demonstrate that hPSC-derived ECCs can generate articular cartilage, which is similar to TMJ condylar cartilage in the microenvironment of the articular cavity.
Activation of the Wnt signalling pathway affects chondrogenic differentiation of ECCs
KEGG analyses of bulk RNA-seq at different induction points showed that the hedgehog and Wnt signalling pathways were remarkably enriched at the last induction point (Fig. 7a). Considering the effects of the small molecules added to the CSSEDF culture medium, we reduced the small molecules one by one to evaluate the chondrogenic differentiation of ECCs. Western blotting analysis showed that the transcription factor SOX9, the condylar chondrocyte anabolism markers COL2A1 and COL1A1, and the catabolism marker MMP13 were obviously upregulated 3 days after the removal of CHIR99021 (Fig. 7b). CHIR99021, as a canonical Wnt signalling agonist, can activate β-catenin, a downstream Wnt mediator55. Obvious downregulation of β-catenin was detected 7 days after the removal of CHIR99021 by western blotting (Fig. 7c). To further confirm the role of Wnt signalling in chondrogenic differentiation, we compared the spontaneous differentiation of ECCs in N2B27 with or without CHIR99021. After adding the Wnt signalling agonist CHIR99021, the chondrogenic transcription factors SOX9 and RUNX2 were decreased, as well as COL2A1 and OPN, while MMP13 was enhanced (Fig. 7d). These results suggest that the activated Wnt signalling pathway can inhibit chondrogenic differentiation of ECCs (Fig. 7e).
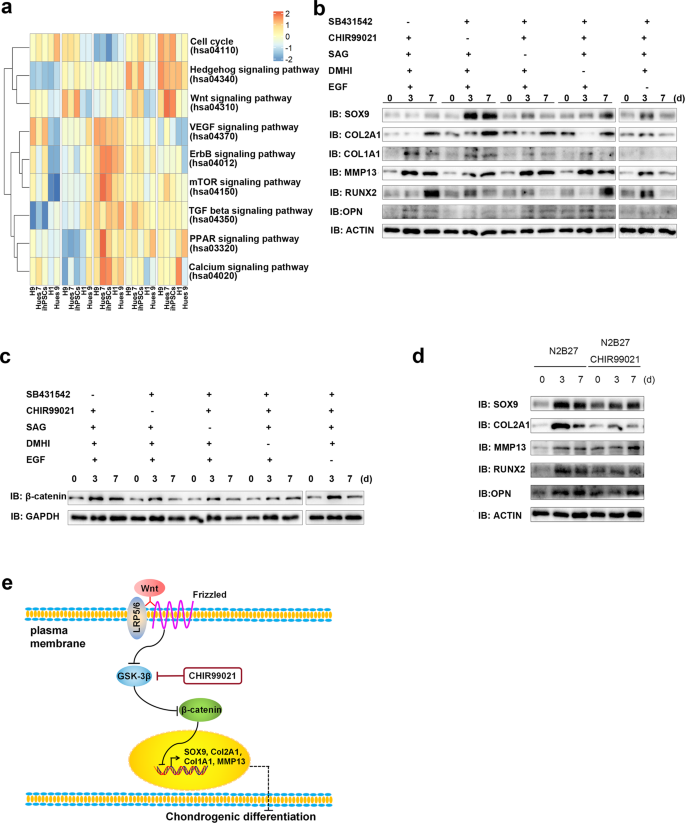
a KEGG analysis of bulk RNA-seq at different induction points. b The transcription factor SOX9, condylar chondrocyte anabolism markers COL2A1 and COL1A1, and the catabolism marker MMP13 were obviously upregulated 3 days after the removal of CHIR99021. c An obvious downregulation of β-catenin was detected by western blotting 7 days after the removal of CHIR99021. d The chondrogenic transcription factors SOX9 and RUNX2 were decreased, as well as COL2A1 and OPN, while MMP13 was enhanced in the presence of CHIR99021 in N2B27. e A scheme for the Wnt signalling pathway involved in chondrogenic differentiation of ECCs.
[ad_2]
Source link